Using Vibration Analysis To Detect Early Failure Of Bearings
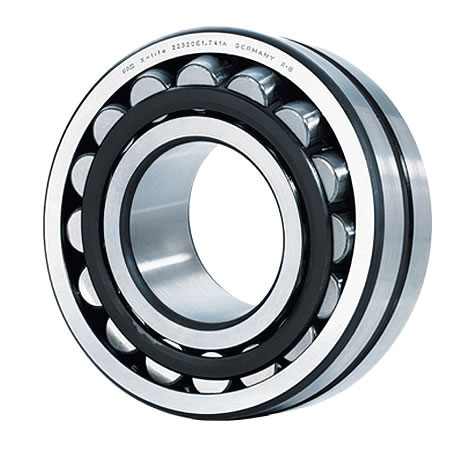
Vibration produced by rolling bearings can be complex and can result from geometrical imperfections during the manufacturing process, defects on the rolling surfaces or geometrical errors in associated components. Noise and vibration is becoming more critical in all types of equipment since it is often perceived to be synonymous with quality and often used for predictive maintenance
By Dr S. J. Lacey, engineering manager, Schaeffler (UK) Ltd
Rolling contact bearings are used in almost every type of rotating machinery, whose reliable operation very much depends on the type of bearing selected and the precision of associated components such as shafts, housings, spacers and nuts.
Bearing engineers generally use fatigue as the normal failure mode, on the assumption that the bearings are properly installed, operated and maintained. Today, because of improvements in manufacturing technology and materials, bearing fatigue life, which is related to sub-surface stresses, is not the limiting factor and accounts for less than 3% of failures in service.
Unfortunately, many bearings fail prematurely in service because of contamination, poor lubrication, temperature extremes, poor fitting, unbalance and misalignment. All these factors lead to an increase in bearing vibration and so condition monitoring has been used for many years to detect degrading bearings before they fail catastrophically, resulting in associated downtime costs and/or significant damage to other parts of the machine.
It is now generally accepted that quiet running is synonymous with the form and finish of the rolling contact surfaces. As a result, bearing manufacturers have developed vibration tests as an effective method for measuring quality. A common approach is to mount the bearing on a quiet-running spindle and measure the radial velocity at a point on the bearing’s outer ring in three frequency bands: 50–300, 300–1,800 and 1,800–10,000Hz. The bearing must meet RMS velocity limits in all three frequency bands.
In the process industries, vibration monitoring is now a well-accepted part of many planned-maintenance regimes and relies on the well-known characteristic vibration signatures, which rolling bearings exhibit as the rolling surfaces degrade. However, in most situations bearing vibration cannot be measured directly and so the bearing vibration signature is modified by the machine structure. This situation is further complicated by vibration from other equipment on the machine, such as electric motors, gears, belts, hydraulics, structural resonance etc. This often makes the interpretation of vibration data difficult, other than by a trained specialist, and can in some situations lead to a mis-diagnosis, resulting in unnecessary machine downtime and costs.
SOURCES OF VIBRATION
Rolling contact bearings represent a complex vibration system whose components (ie rolling elements, inner raceway, outer raceway and cage) interact to generate complex vibration signatures.
Although rolling bearings are manufactured using high-precision machine tools and strict quality controls, inevitably they will have degrees of imperfection and generate vibration as the surfaces interact through a combination of rolling and sliding.
Although the amplitudes of surface imperfections are now in the order of nanometers, significant vibrations can still be produced in the entire audible frequency range (20Hz–20kHz). The level of the vibration will depend upon many factors, including the energy of the impact, the point at which the vibration is measured and the construction of the bearing.
Variable compliance
Under radial and misaligning loads, bearing vibration is an inherent feature of rolling bearings, even if the bearing is geometrically perfect and not, therefore, indicative of poor quality. This type of vibration is often referred to as ‘variable compliance’ and occurs because the external load is supported by a discrete number of rolling elements whose position with respect to the line of action of the load continually changes with time.
Variable compliance vibration is heavily dependant on the number of rolling elements supporting the externally applied load; the greater the number of loaded rolling elements, the less the vibration. For radially loaded or misaligned bearings ‘running clearance’ determines the extent of the load region, and hence, in general, variable compliance increases with clearance.
Geometrical imperfections
Because of the nature of the manufacturing processes used to produce bearing components, geometrical imperfections will always be present to varying degrees depending on the accuracy class of the bearing.
For axially loaded ball bearings operating under moderate speeds, the form and surface finish of the critical rolling surfaces are generally the largest source of noise and vibration.
Controlling component ‘waviness’ and surface finish during the manufacturing process is therefore critical, since it may not only have a significant effect on vibration but also may affect bearing life.
It is convenient to consider geometrical imperfections in terms of wavelength compared with the width of the rolling element–raceway contacts. Surface features of wavelength of the order of the contact width or less are termed roughness, whereas longer wavelength features are termed waviness.
Surface roughness
Surface roughness is a significant source of vibration when its level is high compared with the lubricant film thickness generated between the rolling element–raceway contacts.
Under this condition, surface asperities can break through the lubricant film and interact with the opposing surface, resulting in metal-to-metal contact. The resulting vibration consists of a random sequence of small impulses, which excite all the natural modes of the bearing and supporting structure.
Waviness
For longer wavelength surface features, peak curvatures are low compared with that of the Hertzian contacts and rolling motion is continuous with the rolling elements following the surface contours. The relationship between surface geometry and vibration level is complex and is dependent upon the bearing and contact geometry, as well as conditions of load and speed. Waviness can produce vibration at frequencies up to around 300 times rotational speed but is usually predominant at frequencies below 60 times rotational speed.
For typical bearing surfaces, poor correlation of parallel surface height profiles only exists at shorter wavelengths. Even with modern precision machining technology, waviness cannot be eliminated completely and an element of waviness will always exist albeit at relatively low levels.
As well as the bearing itself, the quality of the associated components can also affect bearing vibration and any geometrical errors on the outside diameter of the shaft or bore of the housing can be reflected on the bearing raceways with the associated increase in vibration. Therefore, careful attention is required to the form and precision of all associated bearing components.
Discrete defects
Whereas surface roughness and waviness result directly from the bearing component manufacturing processes, discrete defects refer to damage of the rolling surfaces due to assembly, contamination, operation, mounting, poor maintenance etc. These defects can be extremely small and difficult to detect but can have a significant impact on vibration-critical equipment and can result in reduced bearing life. This type of defect can take a variety of forms: indentations, scratches along and across the rolling surfaces, pits, debris, and particles in the lubricant.
BEARING CHARACTERISTIC FREQUENCIES
Although the fundamental frequencies generated by rolling bearings are related to relatively simple formulae, they cover a wide frequency range and can interact to give very complex signals. This is often further complicated by the presence of other sources of mechanical, structural or electromechanical vibration on the equipment.
The bearing equations assume that there is no sliding and that the rolling elements roll over the raceway surfaces. However, in practice, this is rarely the case and, due to a number of factors, the rolling elements undergo a combination of rolling and sliding. As a consequence, the actual characteristic defect frequencies may differ slightly from those predicted, but this is very dependent on the type of bearing, operating conditions and fits.
Since most vibration frequencies are proportional to speed, it is important when comparing vibration signatures that data is obtained at identical speeds. Speed changes will cause shifts in the frequency spectrum causing inaccuracies in both the amplitude and frequency measurement. Often with variable-speed equipment, spectral orders may be used where all the frequencies are normalized relative to the fundamental rotational speed. This is called ‘order normalization’ where the fundamental frequency of rotation is called the first order.
Analysis of bearing vibration signals is usually complex and the frequencies generated will add and subtract and are almost always present in bearing vibration spectra. This is particularly true where multiple defects are present.
However, depending upon the dynamic range of the equipment, background noise levels and other sources of vibration, bearing frequencies can be difficult to detect in the early stages of a defect. But, over the years, a number of diagnostic algorithms have been developed to detect bearing faults by measuring the vibration signatures on the bearing housing. Usually, these methods take advantage of both the characteristic frequencies and the ‘ringing frequencies’ (ie natural frequencies) of the bearing.
Raceway defect
A discrete defect on the inner raceway will generate a series of high-energy pulses at a rate equal to the ball pass frequency relative to the inner raceway. Because the inner ring is rotating, the defect will enter and leave the load zone causing a variation in the rolling element–raceway contact force, hence deflections. While in the load zone the amplitudes of the pulses will be highest but then reduce as the defect leaves the load zone resulting in a signal which is amplitude-modulated at inner ring rotational frequency.
A discrete fault on the outer raceway will generate a series of high-energy pulses at a rate equal to the ball pass frequency relative to the outer ring. Because the outer ring is stationary the amplitude of the pulse will theoretically remain the same and hence will appear as a single discrete peak within the frequency domain.
Rolling element defect
Defects on the rolling elements can generate a frequency at twice ball spin frequency and harmonics and the fundamental train frequency. Twice the rolling element spin frequency can be generated when the defect strikes both raceways, but sometimes the frequency may not be this high because the ball is not always in the load zone when the defect strikes and energy is lost as the signal passes through other structural interfaces as it strikes the inner raceway. Also, when a defect on a ball is orientated in the axial direction it will not always contact the inner and outer raceway and therefore may be difficult to detect.
Cage defect
The bearing cage tends to rotate at, typically, 0.4 times inner ring speed, has a low mass and, therefore, unless there is a defect from the manufacturing process, is generally not visible.
Unlike raceway defects, cage failures do not usually excite specific ringing frequencies and this limits the effectiveness of the envelope spectrum. In the case of cage failure, the signature is likely to have random bursts of vibration as the balls slide and the cage starts to wear or deform and a wide band of frequencies is likely to occur.
Other sources of vibration
Contamination is a very common source of bearing deterioration and premature failure and is due to the ingress of foreign particles, either as a result of poor handling or during operation.
By its very nature, the magnitude of the vibration caused by contamination will vary and in the early stages may be difficult to detect, but this depends very much on the type and nature of the contaminants. Contamination can cause wear and damage to the rolling contact surfaces and generate vibration across a broad frequency range. In the early stages the crest factor of the time signal will increase, but it is unlikely that this will be detected in the presence of other sources of vibration.
VIBRATION MEASUREMENT
Vibration measurement can be generally characterized as falling into one of three categories: detection, diagnosis and prognosis.
Detection generally uses the most basic form of vibration measurement, where the overall vibration level is measured on a broadband basis in a range, for example, 10–1,000Hz or 10–10,000Hz. In machines where there is little vibration other than from the bearings, the spikiness of the vibration signal indicated by the Crest Factor (peak/RMS) may imply incipient defects, whereas the high energy level given by the RMS level may indicate severe defects.
Generally, other than to the experienced operator, this type of measurement gives limited information but can be useful when used for trending, where an increasing vibration level is an indicator of a deteriorating machine condition. Trend analysis involves plotting the vibration level as a function of time and using this to predict when the machine must be taken out of service for repair. Another way of using the measurement is to compare the levels with published vibration criteria for different types of equipment.
Although broadband vibration measurement may provide a good starting point for fault detection it has limited diagnostic capability and, although a fault may be identified, it may not give a reliable indication of where the fault is (ie bearing deterioration/damage, unbalance, misalignment etc).
Where an improved diagnostic capability is required, frequency analysis is normally used, which usually gives a much earlier indication of the development of a fault and also the source of the fault.
Having detected and diagnosed a fault, the prognosis (ie what the remaining useful life and possible failure mode of the machine or equipment is) is much more difficult and often relies on the continued monitoring of the fault to determine a suitable time when the equipment can be taken out of service or relies on known experience with similar problems.
Overall vibration level
This is the simplest way of measuring vibration and usually consists of measuring the RMS (Root Mean Square) vibration of the bearing housing or some other point on the machine with the transducer located as close to the bearing as possible.
This technique involves measuring the vibration over a wide frequency range, eg 10–1,000Hz or 10–10,000Hz. The measurements can be trended over time and compared with known levels of vibration or pre-alarm and alarm levels can be set to indicate a change in the machine condition.
Alternatively, measurements can be compared with general standards. Although this method represents a quick and low-cost method of vibration monitoring, it is less sensitive to incipient defects (ie detects defects in the advanced condition) and has a limited diagnostic capability. Also, it is easily influenced by other sources of vibration, such as unbalance, misalignment, looseness, electromagnetic vibration etc.
Frequency spectrum
Frequency analysis plays an important part in the detection and diagnosis of machine faults. In the time domain the individual contributions (eg unbalance, gears etc) to the overall machine vibration are difficult to identify. In the frequency domain they become much easier to identify and can therefore easily be related to individual sources of vibration.
A fault developing in a bearing will show up as increasing vibration at a characteristic frequency making detection possible at a much earlier stage than with overall vibration.
Envelope spectrum
When a bearing starts to deteriorate the resulting time signal often exhibits characteristic features, which can be used to detect a fault. Also, bearing condition can rapidly progress from a very small defect to complete failure in a relatively short period of time; so early detection requires sensitivity to very small changes in the vibration signature.
The vibration signal from the early stage of a defective bearing may be masked by machine noise making it difficult to detect the fault by spectrum analysis alone. The main advantage of envelope analysis is its ability to extract the periodic impacts and the modulated random noise from a deteriorating rolling bearing. This is even possible when the signal from the rolling bearing is relatively low in energy and ‘buried’ within other vibration from the machine.
To request a full copy of the technical paper entitled: ‘An Overview of Bearing Vibration Analysis’, contact the marketing department at Schaeffler on tel: (0121) 351 3833; or email: info.uk@schaeffler.com